For the Public - Research Highlights |
Physics Highlights from the DØ Experiment (1992--1999)
Fermi National Accelerator Laboratory, Batavia, Illinois, U.S.A.
2. THE DØ DETECTOR
For many years, our understanding of nature revolved around four separate, unrelated forces -- gravity (familiar to us all), the electromagnetic
force (involved in everything from the formation of molecules to the pointing of the arrow of a compass northward), the weak force (responsible
for radioactivity), and the strong force (which holds the nuclei of atoms together). Over the past three decades, many experimental and
theoretical advances have led to a coherent and predictive picture of the strong, electromagnetic and weak forces called the Standard Model
(SM). In the SM, the elementary constituents of matter, quarks and leptons, interact through forces, which are transmitted through the exchange
of particles called gauge bosons. Each of these three microscopic forces is described by a gauge theory, in which the interactions are invariant
under changes in the complex phase of the constituent fields at every point in space-time, thus requiring the presence of a spin-1 massless gauge
boson. Gravity remains outside the SM framework.
During the 1960s and 70s, it was recognized that the electromagnetic and weak forces could be described through a unified picture, and the
theory of electroweak interactions was born. A set of four gauge bosons with zero mass was introduced in the SM, together with two pairs of
spin-0 "Higgs" particles, to provide the observed breaking of the symmetry in the underlying electroweak force. As a result of the symmetry
breaking, two of the mediators of the electroweak force, the W and Z bosons, acquire mass, while the photon remains massless. Three of the
Higgs particles are absorbed in giving the W and Z their masses, while the last one remains to be discovered; its mass is not predicted, but can be
inferred in the framework of the SM from precision measurements of other quantities.
The strong force is mediated by a set of eight massless gauge bosons called gluons, and is described by Quantum Chromodynamics (QCD). Of
the matter particles, only the quarks experience the strong force. In the SM, the strong and electroweak interactions are specified separately, but
are not unified. There are compelling reasons to believe that the SM, though remarkably predictive and extremely well tested, is only an
approximate theory to nature. Theories have been postulated that extend the SM, provide unification of the forces, and give deeper understanding
of the Higgs particles. Seeking evidence for the path beyond the SM is the major theme of future experimentation.
According to the SM (see Fig. 2), the particles created at the Tevatron fall into two broad classes: leptons (electron, muon, tau, and neutrinos
associated with each) and hadrons (protons, pions, kaons, etc.), the latter being composed of combinations of the six quarks. The quarks and
leptons are mirrored by their respective antiparticles. In addition, the gauge bosons transmit the fundamental forces; these include the photon
(electromagnetic force), the gluons (QCD strong force), and the W and Z bosons (weak force). Other particles, outside this framework, could
exist and are the subject of many of our searches. Most collisions produce quarks or gluons, which evolve into collimated sprays of hadrons
called jets. These jets usually do not contain leptons, and many of the studies of rare processes -- such as the production of the top quark, W and
Z bosons, or searches for new phenomena -- that would be swamped by backgrounds from copious QCD processes with jets, can be realized
only by using decays of the interesting objects into leptons. Neutrinos and certain newly proposed particles do not interact with matter often
enough to be detected, but can be inferred by an apparent imbalance in momentum conservation. Because of such considerations, the detector
was optimized to measure jets, leptons, and "missing" transverse momentum.
Fig. 2: A table of the elementary particles and force carriers in the Standard Model.
The physics results from DØ rest on the technical achievements of many scientists and engineers. The Fermilab accelerator complex, with its
eight distinct major components, provides high intensity proton and antiproton beams at the world's highest energy (900 GeV for each beam).
These beams collide at two locations in the Tevatron ring, where experiments are performed by the CDF and DØ collaborations. The DØ
experiment contains many sophisticated components, which include not only the particle detectors, but also the electronics needed to select and
digitize events, and the software necessary to monitor the experiment and reconstruct events written to magnetic tape. Although a full description
is not appropriate in this note, it is useful to provide a brief overview of the detector.
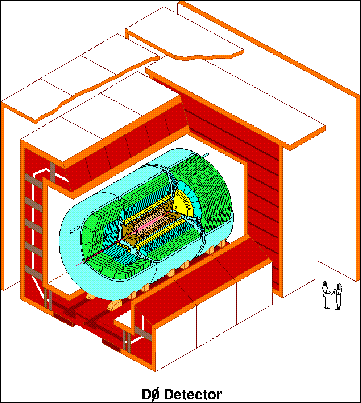
Fig. 3: A schematic view of the DØ detector during Run 1. The tracking chambers near the beam are shown in purple, gray and pink. The calorimeters are shown in yellow, blue, and green. The muon chambers are shown in orange, and surround the iron magnets (in red).
The DØ detector, as it existed in Run 1, is shown in Fig. 3. There were three major subsystems: a collection of tracking detectors extending
from the beam axis to a radius of 30 inches; energy-measuring calorimeters surrounding the tracking region; and, on the outside, a muon detector
that deflected muons using solid iron magnets. The entire detector was about 65 feet long, about 40 feet wide and high, and weighed 5500 tons.
It rested on a moveable platform that permitted detector assembly and commissioning in accessible areas, prior to positioning in the collision hall for operation. The umbilical cord of cables for carrying signals and services followed the detector, and allowed the sensitive electronics for
triggering and digitization to be housed in outer control rooms. The detector was operated around the clock by teams of about six physicists and
technicians, working from the control room, and using the hundreds of available displays to monitor the flow and quality of data. In all, the
detector had over 120,000 channels of individual electronic signals. Some of these were used to take a fast "snapshot" of the properties of an
event, and to decide whether it was a candidate for further study. This "triggering" process proceeded in stages: the first level was completed
within 4 microseconds, before the next accelerator beam-bunches arrived at DØ. A second level of trigger decision followed the digitization of all
information in a farm of dedicated microprocessors. Events that survived this screening process were written to tape and reconstructed in detail
for subsequent analysis.
Figure 4 shows a "typical" event as observed in the DØ detector. The directions of all charged particles were measured in tracking chambers
surrounding the collision point. These detectors relied upon the ionization of a gas caused by the passage of charged particles; the produced
ionization was focussed electrically onto sensors that recorded the amount of charge and its time of arrival, and permitted reconstruction of the
particle trajectory. In addition, the tracking region contained a stack of hundreds of thin foils, called a transition radiation detector. Particles
traversing this detector emitted x-rays with intensity that depended upon their velocity. This device was used to enhance electron identification.
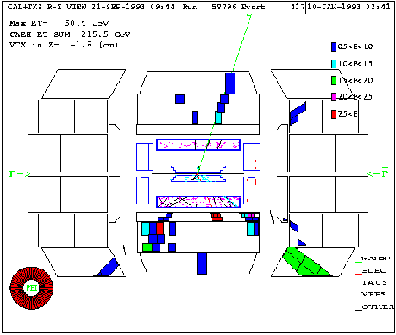
Fig. 4: A side view of "Event 417" referred to in Section 3. The muon track is shown as a green line, the electron track is shown as a short red line, and the two main jet energy depositions in the calorimeters are shown in different colors that represent the energies in the contributing cells.
The energy of most particles (all but muons and neutrinos) was measured in the three calorimeters that surrounded the tracking volume. Each
was composed of a stack of heavy metal plates (uranium, steel or copper) interspersed between gaps containing liquid argon. Particles hitting
upon the calorimeters interacted, yielding secondary particles, which also interacted, leading to a shower of particles that ultimately ended when
all the secondary particles lost energy and stopped. The passage of the full set of showering particles through the argon gaps produced ionization
electrons that were collected on localized electrodes. The observed signal was proportional to the incoming particle energy. The pattern of energy
deposition along the shower was used to distinguish electrons or photons from hadrons. Clusters of deposited energies were used to reconstruct
the jets associated with quarks and gluons.
Muons penetrated the calorimeters, typically without a substantial change in their energy or direction. They were detected in the outer region of
the detector using gas-filled tracking chambers, positioned before and after magnetized blocks of iron. These chambers provided the muon
trajectories before and after the bend in the magnet, and thus yielded the momentum or energy of the muons.
The computer software for DØ was almost completely custom-written. It was required for monitoring and control of the experiment, for the
microprocessors in the trigger system, for controlling the data flow to the ultimate logging to tape, for the reconstruction of particles from the
signals measured in the detector, and for managing the large data samples (70 million events, 3 Terabytes of data) acquired over the run. Special
attention was paid to graphical displays of events and detector performance. Many millions of simulated events were created for study of
detector performance and specific physics processes through "Monte Carlo" programs that mimicked the response of the detector.
< Back Next > - PHYSICS OF THE TOP QUARK
Return to For the Public main page
|